I use spectroscopy and other astronomical techniques to study the formation of planetary systems. In particular, I’m interested in understanding the origin of the diversity of planets and planetary systems. How can the planet formation process produce planets as different as Mercury and Jupiter? When does planet formation produce systems with hot Jupiters, vs. systems with close-in terrestrial planets conducive to life? Which aspects of the planet formation process are universal, and which are instead responsible for the great diversity of planets we see?
To address these questions, I observe the birthplaces of planets – protoplanetary disks. In particular, I use spectroscopy of the gas in disks to study both the chemistry and physics of planet formation.
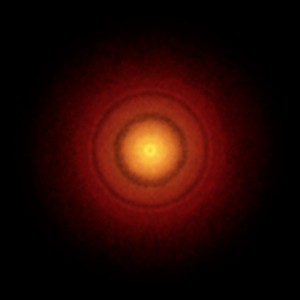
A fabulous image of the protoplanetary disk around TW Hya, obtained with the Atacama Large Millimeter Array. Credit: S. Andrews (Harvard-Smithsonian CfA), ALMA (ESO/NAOJ/NRAO)
Is disk chemistry reset or inherited?
The types of planets that form in any given region of a protoplanetary disk depend sensitively on the local chemistry. Yet with quiescent conditions, only some of the chemistry, in some of the hottest parts of the disk, will have time to come to equilibrium. Therefore, the chemistry depends on the history of the disk. Was the disk mostly quiescent, or was it heated by energetic events? If the latter, how much of the disk was affected? And to what extent has the disk mixed? Studies of solar system materials shows that some parts of the early solar system were heated, other parts weren’t, and materials were mixed over large scales. But what about in other protoplanetary systems?
In 2008, myself and colleagues (Salyk et al. 2008) as well as another team (Carr & Najita 2008) discovered a number of simple molecules in protoplanetary disks with the Spitzer-Infrared Spectrograph (IRS). Since that time, we have determined that detections of these molecules are common (Pontoppidan et al. 2010) allowing us to use them as tracers of disk chemistry. We’ve taken a first cut at understanding the history of disk chemistry by using the Spitzer-IRS spectra to derive molecular abundances and compare them to those expected for the parent cloud out of which the disk forms (Salyk et al. 2011; Pontoppidan et al. 2014). So far, we’ve found evidence that the chemistry in terrestrial planet forming regions has changed significantly since the disk was born. However, these observations are spatially and spectrally unresolved, and when we compare abundances of molecules, we could be comparing unrelated portions of the disk. I am therefore pursuing a variety of ground-based observations to spectrally resolve emission lines from water and other molecules, which will allow us to kinematically locate the molecules in the disk.
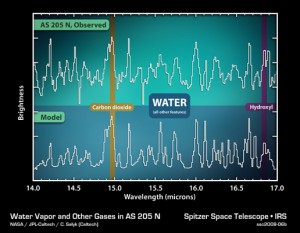
Spitzer-IRS spectrum of the protoplanetary disk around AS 205 N (top) and a molecular emission model (bottom). Most unlabeled features are due to water vapor.
Is the condensation sequence universal?
In the solar system, there is a chemical gradient, with the most volatile-rich planets orbiting furthest from the sun. This is believed to result from an overall heating of the disk, followed by condensation of solids, with composition depending on the ambient temperature – a process known as the condensation sequence (Grossman et al. 1972). I am searching for similar gradients in protoplanetary disks, by beginning to construct radial “maps” of disk chemistry. This is not a trivial task, as it involves measuring chemical abundances using a large number of molecular emission lines across a range of wavelengths, which each trace different parts of the disk. This work is in its early stages, but consists of combining space-based (Spitzer, Herschel) and ground-based (ongoing programs at Keck, Gemini, ALMA) studies.
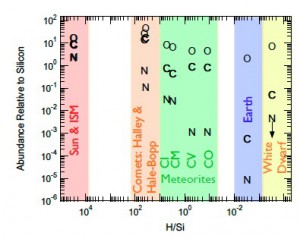
C,N and O abundances of solar system objects and polluted white dwarfs. Notice the increased depletion of C and N in the inner solar system, compared to the outer solar system and the sun. (Credit: Pontoppidan et al. 2014 and references therein).
Where are disk snow lines, and do they influence planet formation?
In addition to affecting the chemistry of planets, disk chemistry can potentially have a much more profound effect on planet formation – it might determine whether a planet becomes a small terrestrial planet, or a much more massive giant planet. This is because wherever a major molecular species (like water, or CO) condenses, it increases the local solid surface density, making it available for the formation of a planetary core. This idea may have originated due to consideration of the solar system, where the water ice condensation front, also called the snow line, was located between the current locations of Mars and Jupiter. However, models have since predicted a strong dependence of planet formation efficiency on solid surface density. Because the snow line location depends on temperature, it also depends on the luminosity of the star and the degree of accretional heating in the disk, and is predicted to evolve with time.
The (surface) snow line can be directly observed in protoplanetary disks by observing water vapor emission from a wide range of excitation states (at a wide range of wavelengths), and mapping its abundance. The water vapor abundance drops precipitously at the snow line radius. First measured in a disk by Meijerink et al. 2009, the second measurement (in a more evolved disk) was performed by graduate student Ke Zhang (Zhang et al. 2013) and showed that the snowline had evolved outwards with time. I am currently analyzing a combination of Spitzer and Herschel molecular spectra to obtain more measurements of snow lines, to determine how they depend on stellar parameters, and on disk evolutionary state.
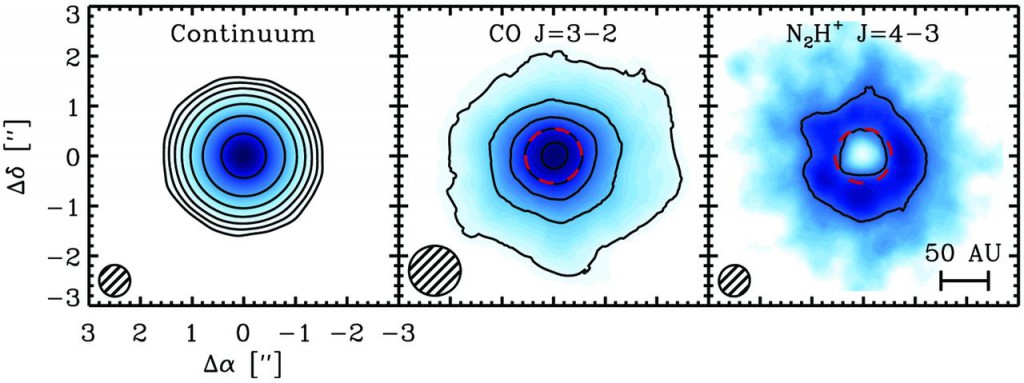
ALMA images showing the location of the CO snowline in the protoplanetary disk surrounding TW Hya (as traced by N2H+) (Credit: Qi et al. 2013). The water snowline can’t be directly imaged, and can only be measured indirectly, via spectroscopy.
Can we observe links between planet formation and C/O ratios?
C/O ratios can have a profound effect on planetary chemistry. If planets form via core accretion, their atmospheric composition will actually reflect the local gas phase C/O, and not the overall C/O of the star (Oberg et al. 2011). The local gas phase C/O is in turn determined by a combination of the C/O ratio of the star, the condensation of molecular carriers of C and O, and transport of C and O bearing molecules. With a team led by Joan Najita, we are investigating whether gas/phase C/O ratios can be observed in disks, and whether these ratios can be correlated with condensation and transport processes. We found an intriguing correlation between HCN/H2O (a proxy for C/O) and disk mass. This could potentially be explained by a relationship between disk mass and the formation and transport of O-rich planetesimals (Najita et al. 2013). We are following up this work with kinematic studies of the HCN and H2O with ground-based spectrographs, to ensure that we are computing abundances in the same part of the disk.
Disk winds
A new sub-class of disks has been discovered, which may straddle the boundary between Stage 1 (embedded disks) and Stage 2 (revealed disks). These disks were identified by their strong, single-peaked line profiles, which require low, non-Keplerian velocities, consistent with a slow disk wind (Bast et al. 2011; Pontoppidan et al. 2011). Although the strong disk wind signatures seem to appear in the evolutionarily youngest disks, there is also some evidence that disk winds may be universal, with wind strengths decreasing as the disk evolves (Pontoppidan et al. 2011; Brown et al., 2013). The exact theoretical mechanism for the wind is not yet known, although it could be related to proposed magnetocentrifugal winds (e.g. Blandford & Payne 1982; Pudritz & Norman 1983). Mass loss rates could be significant, and would occur at planet-forming radii, and could therefore impact the growth of planets. Although the strong disk wind signatures seem to appear in the evolutionarily youngest disks, there is also some evidence that disk winds may be universal, with wind strengths decreasing as the disk evolves (Pontoppidan et al. 2011; Brown et al. 2013). To get a better handle on the velocities and densities of the wind, I observed one disk wind candidate with the Atacama Large Millimeter Array (ALMA), and found evidence for extended, slowly-moving gas, which could potentially arise from a disk wind (Salyk et al. 2014). However, the picture is complicated by the fact that the gas is slower than predicted by wind models. Another possibility is that the gas is being stripped from the disk by a stellar companion, although this hypothesis suffers from plausibility arguments.
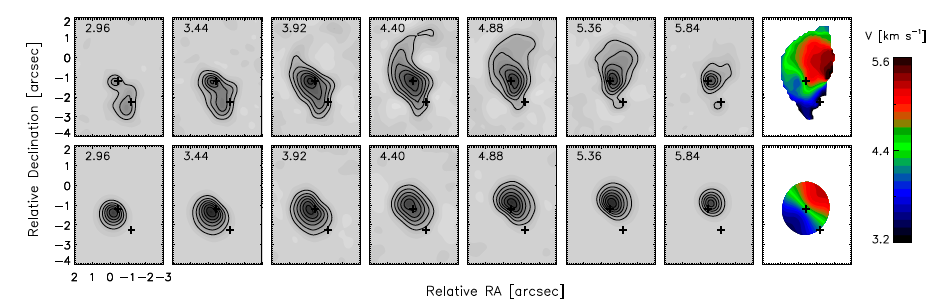
The strange CO velocity field structure of the AS 205 N protoplanetary disk, as seen by ALMA (Credit: Salyk et al. 2014)
Disk evolution and Transition Disks
I also use spectroscopic observations to study disk evolution, including from the embedded stage, to stages in which the disks may already harbor giant planets. At early stages of disk evolution, I have found additional evidence for episodic accretion – the idea that the main phase of stellar formation occurs in short bursts, rather than in a quiescent manner – using an infrared accretion tracer, H I Pf-beta, which can probe disks even behind high levels of extinction (Salyk et al. 2014). At later stages of disk evolution, I showed that transitional disks – disks in which the the planet-forming regions have been cleared of small dust – often still retain significant quantities of gas, consistent with the idea that the clearing is due to the tidal influence of planets (Salyk et al. 2007; Salyk et al. 2009). I have also shown that large dynamical depletions require the influence of planetary systems, rather than single planets (Dodson-Robinson & Salyk 2011).